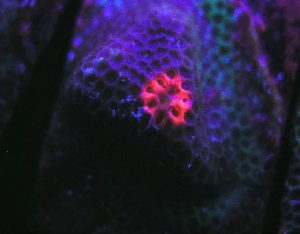
Figure 79. A red fluorescent protein literally glows within a Porites specimen. The pigment was induced during a LED lighting experiment, although the exact reason(s) remain uncertain. It is possible that UV/violet light produced by the LED is responsible, but other possibilities remain. Details of this experiment are discussed below.
Last time, we examined pigments responsible for yellow and orange pigments in corals, as well as briefly discussed light (specific wavelengths) and its potential effects on photoconversion of pigments from one color to another. Before continuing with our discussion of red pigments, this is a good time to take a ‘breather’ and look at several environmental factors and their possible effects on coral coloration.
Environmental Factors and Coloration
It is quite popular to ascribe development of coral pigmentation (or lack thereof) to many factors, including ultraviolet radiation, supplements including iodine, excessive nutrients concentrations, and others. Are there any verified scientific links to certain environmental parameters and induced coloration shifts? We’ll begin our discussion starting with ultraviolet radiation.
Ultraviolet Radiation
Debate of ultraviolet radiation’s (UVR) benefits and/or detriments has occurred since the very beginnings of the reef hobby in North America. It has been touted as necessary for induction of coral coloration and the scientific community’s findings seem to bear this out. So, the question is not one of the necessity of UVR for promotion of pigmentation, it is a question of whether shielding corals from UVR is necessary. The answer will take some detective work involving the spectral output of various lamps and transmission qualities of UVR shields (shields, for our purposes, include various transparent acrylic or ‘plastic’ materials).
Production of UVR by Various Lamps
Ultraviolet energy is classified into three categories. UV-A is radiation just below the violet portion of the visible spectrum and consists of those wavelengths between 320 and 400 nanometers (nm). None of the UV wavelengths are visible to the human eye and are not related, as some hobbyists believe, to the “blueness” of a light source. UV-A (320 – 400 nm) is the least destructive. UV-B wavelengths (280 – 320 nm) are biologically destructive and, through overexposure, cause erythrema (sunburn). The third UV category consists of wavelengths between 200 and 280 nm and is referred to as UV-C. The Earth’s atmosphere absorbs UV-C produced by the sun and therefore is not found naturally. However, UV-C can be produced by artificial means. Arc welding produces UV-C; so do certain specialty electrical lamps (such as those employed by hobbyists for the sterilization of aquarium water). Measurable amounts of UV-C are produced by single-ended metal halide lamps.
Most lamps commonly used by aquarists produce at least some UV-A and UV-B radiation. Generally, metal halide lamps produce greater quantities of UVR than common fluorescent or power compact lamps. Fluorescent lamps (unless specifically designed to do so, and usually accompanied by warnings of UV generation) seldom produce large amounts of UVR, and our discussion of them ends here. On the other hand, UVR production by metal halide lamps is generally not predictable, and its intensity is subject to reflection/focusing by luminaire construction and geometry. In metal halide lamps, ultraviolet radiation usually has peaks at certain wavelengths. For instance, the element mercury is used within arc tubes of metal halide lamps produces a sharp line spectrum at 365nm, while thallium and scandium halides produce peaks at 378nm, 391 nm and 393nm. It would seem an open and shut case: These wavelengths are known to induce coloration (as demonstrated by ‘reddening’ of green pigments in many stony corals), so the logical conclusion is not to shield the lamps at all, but, instead, irradiate the corals with UVR for coloration. This conclusion, as sensible as it seems, could have dire consequences for at least some of your captive corals. Ultraviolet radiation, at wavelengths known to induce coloration and in quantities produced by artificial light sources, has been shown to induce a shut-down of photosynthesis in a process known as dynamic photoinhibition (for a example of photoinhibition by artificial UVR, see Riddle, 2004). The long term effects of dynamic photoinhibition caused by artificial lighting is not known, although we could advance the theory that long-term exposure to UVR could lead to a sort of starvation, where consumption of stored energy reserves until they are exhausted occurs within zooxanthellae, eventually leading to death of symbionts and perhaps the coral animal itself.
Protection of Corals and Zooxanthellae by Natural UV Sunscreens – Not!
Another popular conception in the hobby is that corals and zooxanthellae are protected from UVR by naturally produced sunscreens (known as mycosporine-like amino acids, or MAAs for short). See Table 7 for a concise listing of MAAs – Note that MAA protection is limited to those wavelengths below those known to induce coloration. With this realization, it becomes a practically moot point to discuss the fact that not all zooxanthellae are capable of MAA production. The carry-home point is that natural sunscreens will not offer protection from near-visible (or long-wave UV) wavelengths.
MAA | Max. Absorbance (nm) | Promoted By |
---|---|---|
Mycosporine-Glycine | 310 | UV-B |
Palythine Serine Sulfate | 320 | PAR |
Palythine Serine | 320 | UV-B |
Palythine | 320 | UV-B |
Mycosporine-NMA: Serine | 325 | UV-B |
Mycosporine-NMA: Threonine | 328 | UV-B |
Mycosporine –2 Glycine | 331 | UV-B |
Palythinol | 332 | UV-B |
Porphyra | 334 | UV-B |
Shinorine | 334 | UV-B |
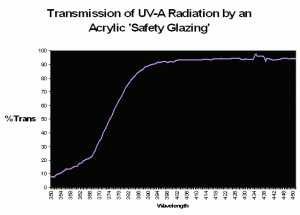
Figure 80. Transmission properties of an acrylic material sold in home improvement stores as a ‘safety glazing’. The wavelength at which there is a 50% reduction in transmitted light (the ‘cut-off’ point) is ~374nm.
Transmission Qualities of Various UV Screens
Some of the first mass-marketed metal halide lighting fixtures included an acrylic ‘screen’ material. It is tempting to think that these were installed to prevent UVR from reaching either the hobbyist, or the inhabitants within the aquarium, but the answer was probably one of utility in that the shield would protect the extremely hot lamp from exploding should it be splashed with water. In the early days of the hobby, there were advocates of subjecting corals to as much UVR as possible, so it seems that the thought of UV shielding were at most secondary, if at all. In any case, these clear acrylic shields were very common on early fixtures, a practice that continues to this day. However, different brands of acrylic have different transmission properties; therefore different acrylics will transmit varying degrees of UVR. To further confound us, the transmission qualities are often determined usually natural sunlight (which can differ radically from the spectrum produced by artificial means. See Figure 81 for ‘bleed-through’ of mercury line spectra through a UV-absorbing ‘safety’ glazing). Further, the ‘cut-off’ point is sometimes listed as a certain wavelength (potentially leading one to believe that no radiation is transmitted below that point), when in reality the determination is made when a fraction (usually 50%) of radiation is transmitted (see Gulko et al., 1995 for further details).
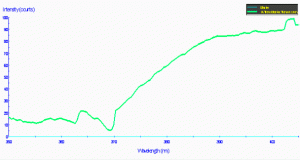
Figure 81. The same material in Figure 80, but showing the transmission qualities of a light source with strong mercury line spectra (this, in fact, from a mercury vapor lamp). This demonstrates that the transmission characteristics are strongly influenced by the spectral quality of the lamp. In other words, UVR can sometimes be only weakened and not eliminated by the shield.
The bottom line – some acrylic ‘shields’ efficiently absorb UV-C and UV-B, but can sometimes transmit fairly effectively many of the UV-A wavelengths know to cause photoconversion in anthozoans. Further, the spectral quality of the light passed through an acrylic shield is highly dependent upon a lamp’s spectral signature.
Shield those lamps for harmful UVR, and you’ll still enjoy brightly colored corals.
As a point of reference, these are UVR and visible line spectra produced by various compounds often found in metal halide lamps:
- Mercury line spectra: 365, 405, 407, 436, 491, 546, 576 and 579nm.
- Thallium line spectra: 378, 535nm.
- Scandium line spectra: 391, 393, 402, 403, 535nm.
- Indium line spectra: 410, 451nm.
Visible Light
It was my initial inclination to report on the effects of visible light in more detail here, but the first stab at compiling the list quickly made me realize that this project is well beyond the scope of this general outline. This is perhaps best, for now, since this project can include the effects of visible light on fluorescent proteins and chromoproteins (chromoproteins will be the subject of the next article in this series). See the third installment of this series for a synopsis of wavelength and production of fluorescent proteins.
Apparent Water Color and its Effect on Light Transmission
Water color is important to aquarists because it is usually a yellowish tint resulting from an accumulation of organic compounds from decaying materials. This yellow color can absorb, to varying degrees, many of the wavelengths known to induce photoconversion.
‘Apparent water color’ differs from ‘true water color’ in that the true color is determined after filtration (removal) of suspended solids. This is usually not practical for most hobbyists. Therefore, ‘apparent color’ is determined visually or with a spectrometer on a sample grabbed from an aquarium. Water color is influenced by pH, but since we’re dealing with a fairly narrow acceptable pH in a reef tank, its effects are most likely minimal.
Fortunately, management of water color is not a difficult task, and protein skimmers combined with use of activated carbon can maintain very low water color (i.e., 2). See Bingman, 1995 for the full story on activated carbon and water clarity (this reference is listed at the end of this article). Judicious use of ozone (generally used in tandem with activated carbon) will aid in the removal of yellow compounds and increase the transmission of UVR, violet and blue wavelengths.
The acceptable protocol (APHA, 1998) for measurement of the color of water is one where a 500 milliliter grab or composite sample is obtained in a clean glass or plastic container, and analyzed as soon a possible (samples not analyzed immediately should be refrigerated and stored up to a maximum of 48 hours. Report the sample pH). Color can be determined with a hand-held color comparator or with a spectrometer and is reported as platinum-cobalt color units ranging from 0-100.
It has been my experience that even the most yellow of aquarium waters rarely exceed 15 standard color units, thus limiting the usefulness of visual comparators; it is obvious that serious works with water coloration require a calibrated colorimeter or spectrometer as well a pH meter.
Molecular Oxygen
GFP from the jellyfish Aequorea victoria requires molecular – “free” – oxygen during its transition from a colorless protein to a fluorescent green one. Once matured, presence of oxygen does not affect fluorescence (Tsien, 1998). However, Elowitz, 1997, suggests transition of this particular GFP to ‘super red’ occurs under anoxic or anaerobic conditions.
It is unknown at this point how corals, in artificial environments, react to low oxygen concentrations we might expect to see at night and probably under conditions of very low flow, where the coral/symbiont’s combined respiration could possibly create anoxic conditions. I have some experiments planned for testing the effects of low oxygen in aquarium conditions, but these are still in their infancy. We have a lot to learn.
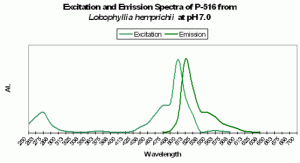
Figure 82. Excitation and emission spectra from a stony coral at the relatively low pH of 7.0. We would expect this low pH to occur (possibly) only in darkness, due to respiration and lack of photosynthesis.
pH
As we have seen, the respective concentrations of hydrogen ions and hydroxyl ions (pH) are known to cause color shifts in some pigments. Could photosynthetically-driven changes of pH cause intensification of pigment color? Fortunately, there is some related data available. Fitt et al., 1995 describe diurnal shifts of pH ranging from ~8.1 (day) to ~7.2 (night) within the ‘blood’ of giant clams Tridacna derasa and T. gigas. This shift is thought due to the effects of photosynthesis on the concentrations of inorganic carbon within the hemolymph. Similar pH shifts have been noted in zooxanthellae cultures from Tridacna crocea (D’Elia et al. 1983). Photosynthesis within the red fleshy alga Gracilaria conferta causes shifts to as high as 9.4 on the surface of the thalli (Israel and Beer, 1992). It is tempting to think that zooxanthellae photosynthesis could cause modulations in internal and surface tissues pH of corals, and could account for some of the reports of pigment intensification during ‘daylight’ hours (Steve Tyree, personal communication).
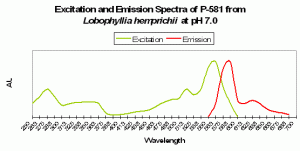
Figure 83. P-581, the mature form of P-516 (seen in Figures 81 and 82). Notice, in particular, how low pH has apparently caused a shift in the emission spectrum – it is no longer 581nm!
pH is known to affect the color of water (see the notes on water coloration, above). It seems evident that coral coloration is affected by pH values as well.
Temperature
Fluorescent pigments from tropical anthozoans tend to mature quicker at higher temperatures (>30°C – 86ºF!). It is believed that these temperature-dependent rates may be an evolutionary adaptation to warmer tropical waters (Terskikha et al., 2002). This should not be interpreted as a recommendation for high temperature within an aquarium! Just be aware that temperature plays a role in the biochemical transformations of fluorescent proteins. Even short-lived high temperature excursions can cause loss of host coloration in at least some corals. Dove et al., 2006 found Montipora monasteriata specimens lost up to 75% of their purple protein content after exposure to a temperature of 32ºC (89.6ºF) for only 6 hours (light intensity during these experiments never exceeded 650 µmol·m²·sec, or ~33,000 lux). These specimens were collected in shallow water – 3 to 5m – where they naturally experienced maximum light intensity exceeding 1,000 µmol·m²·sec; ~50,000 lux). Other FPs (notably P-611 from E. quadricolor) are sensitive to high temperatures as well, and denature (“break down”) at temperatures approaching that of the human body, making them useless for biomedical research.
Salih et al. (2000) observed that colorful corals were less susceptible to severe bleaching events than their ‘zooxanthellae-brown’ neighbors, and concluded that colorful pigments likely play a photoprotective role. However, in 2006, Fabricius reported that lightly colored corals absorbed less infrared radiation (heat) than darkly colored specimens and it is possible that Salih’s fluorescent corals gained less heat, resulting in ‘bleaching resistance.’
Iodide and Other Halides
Supplemental iodine addition to reef tanks has been popular for a number of years. Some crustaceans require it and, anecdotally, so do Xenia and other soft coral genera (although it is unclear if the element is beneficial to corals for its disinfecting qualities, or some other biological reason). An interesting observation has been an apparent link between iodine dosage and ‘false coral’ coloration (Delbeek and Sprung, 1994). There is now some evidence, albeit scant and a bit of a stretch to make the ‘aquarium connection’, that iodide can play a part in transitioning apparent coloration in coral tissues. Certain genetically-engineered variants of YPF (yellow fluorescent proteins, including YFP-H148Q and YFP-H148G) are affected by halides (fluoride, iodide, chloride (!) and bromide) at lower (acidic) pH levels. The ‘natural’ pocilloporan Rtms5 (from the stony coral Montipora efflorescens) is relatively insensitive to iodide additions (Wachter and Remington, 1999). This is interesting work, and just the beginning of our journey in understanding the role halides play in anthozoan fluorescence and coloration.
Nitrate
Nutrient control within an aquarium is essential and can be performed in a number of ways (including removal through biological, chemical or mechanical means). Perhaps the best reason for nutrient management is that of algae control. By extension, many hobbyists have linked ‘high’ nutrient levels to poor coloration or ‘browning’ of corals; that is; nitrogen fertilization increases the density of zooxanthellae and masks coloration (see below).
Relatively low nitrate concentrations (~10 mM and higher, or about 0.62 mg/l NO3) are known to inhibit fluorescence of some genetically-modified fluorescent proteins (Wachter and Remington, 1999). But what of naturally occurring proteins?
We should remember that nutrient concentrations within coral tissues containing zooxanthellae could be lower than those of the ambient water. As an observation, seawater off the Kona (leeward side of the Big Island of Hawaii) coast contains relatively high amounts of nitrate and coloration of many corals is still quite vivid. Much work is needed in this area before we can determine the effects of nitrate (if any) on coral FPs and pocilloporans. However, see comments below.
Aquarium Mythology: Nutrient Levels Increase Number of Zooxanthellae, Mask Color, and Increased Light Lowers Zooxanthellae Density
While zooxanthellae density could possibly mask host pigmentation, the notion that this occurs consistently is incorrect. This statement is based on observations made in one of the display tanks at the Natural Energy Laboratory (NELHA) in Kailua-Kona, Hawaii.
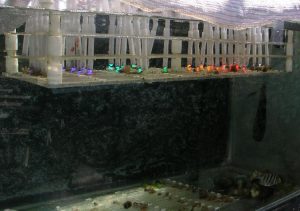
Figure 84. A simple experiment investigating the potential effects of narrow bandwidth light (produced by LEDs) on coral pigmentation. Note the shade cloth draped over the top of this outdoor aquarium.
NELHA provides to its customers warm seawater pumped from a few meters’ depth as well as cold, nutrient rich from great depth. This blurb from NELHA’s website sums it up nicely: “The deep seawater also has significantly elevated levels of inorganic nitrates, silicates and phosphates compared to the nutrient deficient surface water. These nutrients are necessary for sustained growth of microalgae and macroalgae. All parameters of both the surface and deep seawater indicate low long-term variability. This characteristic provides reliability to the users of the seawater.” (See www.nelha.org for further details). A coral tank was maintained on site with a flow-through of ‘surface’ water and a slight trickle of cold water to maintain a reasonable temperature during the day. Thus, the corals were exposed to a nutrient-enriched environment. For more information on this experiment, see Riddle, 2003.
The purpose of this display was two-fold – one, to demonstrate stony coral propagation, and secondly, to investigate the effects of different spectra provided by LED lamps on coral coloration. See Figure 85.
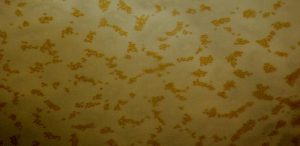
Figure 85. Zooxanthellae (appearing as reddish-brown dots) within a brown Pocillopora fragment. This specimen was grown on a microscope slide over the course of a couple of months, and ‘plated out’ to form a thin semi-transparent living slide.
The reddish pigment is believed to be a non-fluorescent chromoprotein called ‘pocilloporan’.
Coral Shifts (Undetermined Origins)
Some color shifts are of undetermined origin. Todd et al. (2002) report color shifts of green to orange-red with increasing water depth in the stony corals Favia speciosa and Diploastrea heliopora. Observations of Kelmanson and Matz (2003) suggest the same color transitions under similar conditions are seen in the stony coral Montastraea cavernosa, with red colonies being more abundant in deeper (12-14m) waters.
The orange coloration could be due to either an orange-fluorescing protein, or phycoerythrin (see Part III of this series for notes on the symbiotic relationship between zooxanthellae and cyanobacteria).
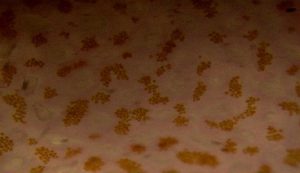
Figure 86. Same spot, same coral as shown in Figure 85, but after a few weeks’ exposure to more intense light. Note the pinkish color of the tissue and, more importantly, the density and color of zooxanthellae – their numbers are about equal to those seen in Figure 85, and their coloration isn’t changed much. In the case of this Pocillopora, increased light did not result in a lower density of symbiotic algae that would mask coloration. Similar color shifts have been noted when Pocillopora specimens have been exposed to narrow bandwidth light generated by a blue LED.
Red Fluorescence
Fluorescence is not restricted to visible wavelengths and it can occur beyond the range of the human eye. However, our discussion of fluorescent pigments ends with those visibly red pigments.
Red fluorescent pigments that maintain their integrity and higher temperatures and a range of pH’s are of great interest to the biomedical field as use as bio-markers. Relatively few anthozoan red fluorescent proteins have been described when compared to green fluorescent proteins. Though limited in number, red fluorescent proteins (RFPs) are fairly abundant and make for some of the most desirable specimens when present in corals, especially when combined with other pigments.
Reddish fluorescence is seen in many anthozoans, including the ‘false’ coral Discosoma, and stony corals Goniastrea, Lobophyllia hemprichii, Lobophyllia hataii, Cyphastraea microphthalma, Acropora spp. (Pieribone and Gruber, 2005), Montipora digitata (Salih et al. 2000),Montipora monasteriata, Pocillopora damicornis, Acropora horrida, Porites murrayensis, Acropora aspera (Dove et al., 2001), Porites astreoides (Mazel, 2003), and Plesiastrea verispora (Salih, 2004) and likely many others. See Table 8.
Pink coloration could be the result of a low-level red fluorescence, or simply indicate the presence of a reflective chromoprotein. It is worth noting that Shibata (1969) reports the absorbance of a fluorescent pigment found in a pink Acropora specimen. Excitation (in visible wavelengths) is maximal at ~560nm (see Figure 87). No emission spectrum is reported by Shibata, but it seems fairly certain that the fluorescent band falls into either a pinkish or red category.
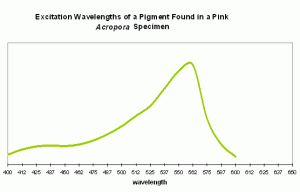
Figure 87. Excitation wavelengths for a pink coloration found in an Acropora specimen. After Shibata, 1969. Maximum absorption at ~560nm is close to line emission spectra of lamps containing the element mercury.
Pigment | Emission | 2 | 3 | Excitation | 2 | 3 | Found in: | Reference |
---|---|---|---|---|---|---|---|---|
P-610 | 610 | * | * | 570 | * | * | Montipora monasteriata | Dove et al., 2001 |
P-611 | 611 | * | * | 559 | * | * | Entacmaea quadricolor | Wiedenmann et al., 2002 |
P-620 | 620 | * | * | 440 | * | * | Montipora sp. | Kogure et al., 2006 |
P-620 | 620 | * | * | 490 | * | * | Porites astreoides | Mazel et al., 2003 |
P-620 | 620 | * | * | * | * | * | Plesiastrea verispora (blue morph) | Salih et al., 2004 |
P-620 | 620 | * | * | * | * | * | Plesiastrea verispora (green morph) | Salih et al., 2004 |
P-625 | 625 | * | * | 570 | * | * | Pocillopora damicornis | Dove et al., 2001 |
P-625 | 625 | * | * | 575 | * | * | Acropora horrida | Dove et al., 2001 |
P-625 | 625 | * | * | 570 | * | * | Porites murrayensis | Dove et al., 2001 |
P-630 | 630 | * | * | 576 | * | * | Acropora aspera | Dove et al., 2001 |
P-685 | 685 | * | * | Violet/ Blue | Red | * | Chlorophyll – common to healthy hermatypic corals | Multiple references |
And now, an examination of a few of the known red-fluorescing pigments.
P-610
Host: Montipora monasteriata
- Excitation: 570nm
- Emission: 610nm
- Stokes shift: 40nm
- Reference: Dove et al., 2001
- Comments: This particular coral was purple in natural light – Intact coral tissue absorbs orange light most intensely at 579nm (data not shown).
P-611: Mature Form of P-520
Host: Entacmaea quadricolor
- Excitation: 559nm; Shoulder at 525nm.
- Emission: 611nm
- Stokes shift: 52 nm
- Reference: Wiedenmann et al., 2002; Nienhaus et al., 2003
- Comments: Wiedenmann received this anemone as a gift from the Ulm (Germany) Association of Seawater Aquarists. The fluorescent quantum yield is 0.45. As with many fluorescent colors found in marine invertebrates, green fluorescence matures to red fluorescence. Even when completely matured, there is still residual green fluorescence estimated to be about 1% of the red emission. Chemical oxidation is responsible for color conversion – light is not a factor as the conversion can occur in darkness. This GFP is sensitive to temperature and is not suitable as a marker protein at human body temperature. Intolerance of higher temperatures gives aquarists a ‘heads up’ for maintenance of host fluorescence – aquarium temperatures not exceeding about 28ºC (~82º F) should be suitable.
Figure 89. A red pigment from the stony coral Montipora. Note the huge Stokes shift – 180nm! After Kogure et al., 2006.
P-616: Commercially Available as ‘Keima-Red’
Host: Montipora sp.
- Excitation: 440nm
- Emission: 620nm
- Stokes shift: 180nm
- Reference: Kogure et al., 2006
- Comments: This fluorescent protein is stable in aerobic conditions and higher temperatures (37ºC). This pigment has been cloned and is commercially available under the trade name Keima-Red.
P-620
Host: Porites astreoides
- Excitation: 490nm
- Emission: 620nm
- Stokes shift: 130nm
- Reference: Mazel et al., 2003.
- Comment: See Figure 91.
P-625
Host: Pocillopora damicornis
- Excitation: 570nm
- Emission: 625nm
- Stokes shift: 55nm
- Reference: Dove et al., 2001
- Comments: Coral appears pink when viewed in natural light. Tissue absorbs light most intensely at 560nm (data not shown, but see Figure 88 for information on an Acropora pigment absorbing light at 560nm).
Host: Acropora horrida
- Excitation: 575nm
- Emission: 625nm
- Stokes shift: 50nm
- Reference: Dove et al., 2001
- Comments: Coral polyps of A. horrida are often devoid of zooxanthellae – and fluorescent pigmentation (Salih et al., 1999). Fluorescent granules are concentrated above zooxanthellae layer in ‘strong’ light. Tissue absorbs light most strongly at 579nm (data not shown). This coral appeared blue in natural light conditions.
Host: Porites murrayensis
- Excitation: 570nm
- Emission: 625nm
- Stokes shift: 55nm
- Reference: Dove et al., 2001
- Comments: Appears to be the same P-625 found in Pocillopora damicornis (above). Coral tissue and zooxanthellae combined absorbed light most strongly at 576nm (data not shown). This coral appears purple in natural light (it is possibly in combination with other fluorescent pigments. See below for a brief introduction to color mixing).
P-630
Host: Acropora aspera
- Excitation: 576nm
- Emission: 630nm
- Stokes shift: 54nm
- Reference: Dove et al., 2001
- Comments: This coral appears blue in natural light; P-630 is in combination with at least one other pigment, probably a non-fluorescent chromoprotein. Maximum coral tissue/zooxanthellae combination absorbance is 580nm (data not shown).
P-645 or HcRed
P-645 is a genetically-engineered fluorescent mutant of the chromoprotein from the anemone Heteractis crispa (Verkusha and Lukyanov, 2004). It is not known to occur naturally.
P-685 Chlorophyll Fluorescence
Chlorophyll a (Chl a)fluorescence is in the deep red portion of the spectrum but is generally not visible to the unaided eye (its fluorescence is sometimes visible given proper excitation in darkened conditions). Of course, Chl a is a major photosynthetic pigment in zooxanthellae. Chlorophyll fluorescence is one method of ‘dumping’ excess energy away from Photosystem II reaction centers, thereby acting as a ‘pressure relief valve’ during periods of intense illumination and preventing destruction of the photosynthetic apparatus. See Figures 94, 95 and 96 for more information on chlorophyll absorbance, emission and color mixing.
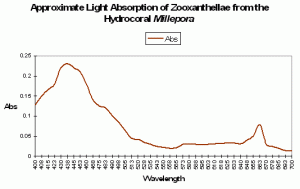
Figure 93. An action spectrum for zooxanthellae containing pigments chl a, chl c2 and peridinin. After Schonwald et al., 1986.
Mixing of Colors
Color mixing of three primary colors, as we know, can produce an astounding array of apparent colors. And as any painter can tell you, subtle changes in the composition of the mix can produce a profoundly different color. Apparent color of pigmented color tissues is even more complicated since fluorescent and reflective pigments can combine to produce color. In addition, the excitation wavelengths, quantum yield of fluorescent pigments and spectral quality of the excitation light source combine to make prediction of color a truly mind-boggling exercise. However, a simple exercise using only three different colors (seen in Figure 97) gives us an idea of the broad range of colors possible when two pigments are mixed, or when a single pigment is in transition from green to red (or vice versa).
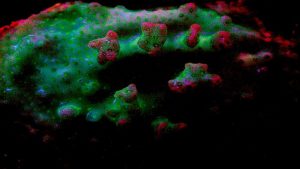
Figure 94. A macro shot of a Pocillopora damicornis. The red coloration is believed from the chlorophyll content within the captive zooxanthellae. Notice how the blue-green/red transition can appear purple. Due to the possible presence of a non-fluorescent chromoprotein, the coral appears pink in daylight, although further work is needed to differentiate chromoprotein spectral qualities from those of fluorescent pigments.
Top row, left to right: Mixing of a blue-green pigment (first column) and ‘pure’ red (last column).The second column in the top row is a 66/33% mixture of the blue-green and red pigments; the second column is a 50/50 mix; column 3 is a 33/66% mixture.
The same percentages apply to Rows 2 and 3.
These color mixtures assume an equal fluorescent quantum yield for all colors. Imagine the staggering number of combinations when 10 or 12 pigments are in play.
This ends our discussion of fluorescent pigments for now. Next time, we’ll begin an investigation of the non-fluorescent chromoproteins found within the anthozoans of our captive reefs.
Those interested in doing so can reach me at [email protected].
See Part 1 of this series for a listing of references. The references listed below have been added since the initial compilation.
Additional References
- American Public Health Association (APHA), 1998. Standard Methods for the Examination of Water and Wastewater. Edited by L. Cleceri, A. Greenberg and A. Eaton. Washington, DC.
- Banaszak, A., T. LaJeunesse and R. Trench, 2000. The synthesis of mycosporine-like amino acids (MAAs) by cultured, symbiotic dinoflagellates. J. Exp. Mar. Biol. Ecol., 249: 219-233.
- Bingman, C., 1995. The effect of activated carbon treatment on the transmission of visible and UV light through aquarium water. I: Time-course of activated carbon treatment and biological effects. Aquarium Frontiers 2(3): 4,5,16-19.
- Dunlap, W.C. and B.E. Chalker, 1986. Identification and quantification of near-UV absorbing compounds (S-320) in a hermatypic scleractinian. Coral Reefs, 5:155-159.
- Kuffner, I.B., M.E. Ondrusek and M.P. Lesser, 1995. Distribution of mycosporine-like amino acids in the tissues of Hawaiian scleractinia: a depth profile. In: Ultraviolet Radiation and Coral Reefs. D. Gulko and P.L. Jokiel, Eds. HIMB Tech. Report #41.
- Riddle, D., 2003. Effects of narrow bandwidth light sources on coral host and zooxanthellae pigments: http://advancedaquarist.com/issues/nov2003/feature.htm
- Riddle, D., 2004. Playing with poison – Ultraviolet radiation: http://advancedaquarist.com/issues/aug2004/feature.htm
0 Comments