The aquarium containing marine invertebrates evolved as an extension of the ‘fish-only’ marine aquarium that has been popular since the 1960’s. Hence, many of the challenges (such as ammonia control, metal toxicity, etc.) of marine tanks had been overcome by the time reef keeping came into existence. However, successful maintenance of photosynthetic invertebrates presented a new set of challenges. The reef aquarium hobby has made many advances over the last 20 years, and much of this has been due to willingness of hobbyists (read tinkers) to think creatively when solving problems. Still, some coral specimens and species are difficult to maintain in captivity.
The issues of lighting and water motion have likely been resolved as much as possible with strictly anecdotal observation. This short article reports results of experiments using some rather sophisticated equipment that allow us to ‘ask’ – and corals to ‘answer’ – some important questions.
Part I: Spectral Experiment – Effects of Metal Halide Lamp Spectral Qualities on Zooxanthellae Photosynthesis in Photoacclimated Fungia Corals: The Red Light Theory
An article in the February 2002 AAOL (“Spectrum or Intensity?” – Riddle and Oliazola) reported results of an experiment designed to determine the effects of different metal halide lamps on rates of photosynthesis in the Hawaiian stony coral Fungia scutaria. The outcome of this experiment suggested there is little difference in the rates of photosynthesis of sunlight-adapted corals upon initial exposure to different lamp spectra (high Kelvin – 20,000 K – and lower Kelvin – 4,000 K- lamps) if light intensity is the same.
The results raised many questions, and created healthy debate. In order to address some of the issues that followed the article’s publication, it was decided to conduct new procedures to determine the effects of spectral quality on zooxanthellae photosynthesis. Two ‘Mushroom’ coral ( Fungia scutaria) specimens were allowed to adapt to different lamp spectra (10,000 K and ~50,000 K) for a 30-day period. Analyses of quantum yields and relative Electron Transport Rates (ETRs) were calculated via Pulsed Amplitude Modulation (PAM) fluorometry for each coral under different lamp spectra. The results suggest that rates of zooxanthellae photosynthesis were essentially the same within two photoacclimated corals. A theory (involving red light) for observed similarity of ETRs is discussed in the following article.
Introduction
Photosynthesis is a biochemical process that even today is not fully understood; however, there are processes and concepts that are well established and accepted. This brief introduction will review these concepts. Note: To avoid redundancy, readers are encouraged to review the report of initial experiments published in the February 2002 Advanced Aquarist (available in the Archive section). This article describes various technical aspects pertinent to both sets of experiments.
Corals containing symbiotic dinoflagellates (zooxanthellae) of the genus Symbiodinium are dependent upon light energy to power photosynthesis. The photochemical apparatus within a zooxanthella is similar to those found in other oxygen-evolving photosynthetic algae, plants and symbiotic creatures. There are two photosystems involved, called Photosystem One (PS I) and Photosystem Two (PS II). Light-absorbing pigments called photopigments harvest light energy. Chlorophylls (chlorophyll a and chlorophyll c2 ) are the most recognized photopigments of zooxanthellae, but others are involved as well, and these are called accessory (or antennae) pigments. In corals, accessory pigments include peridinin and beta-carotene. Chlorophylls and accessory pigments can channel the energy they harvest to both photosystems, although it is possible for an accessory pigment (such as beta-carotene, peridinin, etc.) to transfer its collected energy almost exclusively to one photosystem (PS I in the case of beta-carotene).
In general terms, PS II is associated with the splitting of water molecules to form molecular oxygen; PS I involves transfer of energy to the Calvin Cycle, where inorganic carbon is converted to simple sugar. In one of the quirks of scientific nomenclature, photosynthesis begins with Photosystem II. If PS II fails, the electron flow from PS II to PS I stops, and the entire photosynthetic process grinds to a halt.
Quantum yield (or photosynthetic yield) describes the number of events that occur per photon absorbed by the photosystem. In this article, we report relative quantum yield, which is a comparison of chlorophyll fluorescence signals and light intensity falling upon the coral’s surface. In other words, quantum yield equals moles of chlorophyll fluorescence divided by moles of light energy available to Photosystem II.
Procedure
Two pre-production samples of metal halide lamps were selected for use in this experiment. Both were 175-watt versions, but were of different Kelvin ratings. One lamp was advertised to have a Kelvin rating of about 10,000; the other lamp did not have a Kelvin rating from the manufacturer, who advertised the lamp only as ‘sky blue’ (similar lamps have a true – not advertised – Kelvin rating of 40,000 – 50,000 K). For ease of reference, these lamps will be called Lamp 10K and Lamp 50K. An Ocean Optics spectrometer was used to measure the Spectral Power Distribution (SPD). Figures 1 and 2 demonstrate graphical SPDs of these two lamps (both spectrometer scans were made with the lamps’ Photosynthetic Photon Flux Density (PPFD) at 24 μmol/m2/sec; hence the comparisons are more meaningful).
All experiments were conducted with corals held at the West Hawaii Explorations Academy (WHEA) within the Natural Energy Laboratory Hawaii (NELHA) complex in Kailua-Kona. These corals were legally collected under a scientific permit from the Hawaii Department of Aquatic Resources. Two Fungia scutaria specimens were selected from a 300-gallon open system using natural sunlight as the light source (attenuated by shade cloth to a maximum of about 17% intensity, or a maximum of ~350 micromol-m2-sec.).
These two corals had distinctive markings and, to make our lives easier when handling them during experiments were given nicknames – ‘Greta’ and ‘Smiley.’ These corals were transferred to a 100-gallon Rubbermaid tub with a water flow throughput of about 5 gallons per minute. (See Figure 3 for a drawing of the experiment’s setup). A Coralife luminaire containing Lamps 10K and 50K was placed atop the tub and 3mil heavy duty black plastic was taped to the light hood and extended over the edge of the tub to exclude any natural light. An acrylic ‘splash guard’ attenuated ultraviolet radiation to extremely low levels in order to prevent potential alteration of zooxanthellae densities (Kinzie, 1993). A serpentine light trap was placed in the middle of the luminaire – this allowed air circulation through the luminaire while isolating the two light sources. A black plastic partition extended from the top of the tank to a false bottom of eggcrate material and divided the Rubbermaid trough into two equivalent sections with distinctly different light fields. Photoperiod was 14 hours per day, and controlled by a small electric appliance timer. Lengths of PVC elevated the eggcrate and the corals were placed upon the eggcrate, which was marked with a waterproof marker to indicate the corals’ respective positions (so they could be moved to their original positions should they decide to ‘walk.’ As it happened, the corals did not move during the experiment). The coral nicknamed Greta was photoadapted to the low Kelvin ‘Lamp 10K’ lamp while ‘Smiley’ was adapted to the much bluer ‘Lamp 50K’ lamp. Each coral was allowed to adapt for a period of 30 days to the particular light spectrum of the lamp above it.
At the end of this period, the corals were removed from the tub and transferred to a 10-gallon aquarium within a darkened, air-conditioned room at the Natural Energy Laboratory. After a 30-minute dark adaptation period (to allow PS II reaction centers to ‘open’), a coral was placed in a shallow plastic basin. A small air pump aerated the basin and also provided water circulation (an important consideration – see Part 2 of this article). Water temperature in this basin remained relatively constant during the time required to take the measurements. Temperature difference and its effects on quantum yield were not considered a significant factor (see Iglesias-Prieto, 1997). The fiber optic cable of a Walz Pulsed Amplitude Modulation (PAM) fluorometer measured the Photosynthetic Yield of zooxanthellae on a particular spot of each coral under each of the two metal halide lamps. Measurements were made in accordance with recommendations made by Schreiber (1997). The Li-Cor quantum sensor was placed next to each coral so that PPFD would be standardized under each metal halide lamp. Measurements were made at each of these light intensities: 25, 50, 75, 100 and 150 micromol-m2-sec (these light intensities are commonly found in reef aquaria, and are assumed to be sub- saturating). Three measurements of Quantum Yield were taken at each light intensity for both corals under each of the two lamps. Relative Electron Transport Rate (ETR) was calculated using the formula ETR = Quantum Yield multiplied by the Photosynthetic Photon Flux Density (PPFD, reported as μmol/m2/sec) absorbed by the photosystem (as in the first set of experiments, we report Relative ETR since we did not measure the amount of light actually absorbed. We made the assumption that the zooxanthellae pigments did not change in the few minutes required to measure the quantum yield). The results were entered into an Excel worksheet. Since no photoinhibition was apparent from the results (as would be indicated by a drop in the relative ETR), linear regression was used to fit the data.
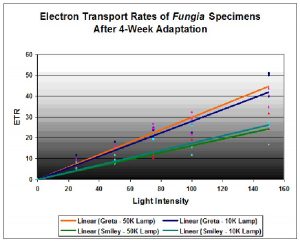
Figure 4. Results of the photoacclimation experiment. Fungia ‘Greta’ was acclimated to the 10,000K lamp; Fungia ‘Smiley’ adapted to the 50,000K lamp.
Results
The results are illustrated in Figure 4. They indicate that the Fungia nicknamed Greta had a higher relative electron transport rate when illuminated by both Lamp 10K and Lamp 50K than the Fungia nicknamed Smiley. However, there is little difference of individual coral/zooxanthellae ETR when illuminated by either Lamp 10K or Lamp 50K. The results suggest that even after photoadaptation, spectral quality played a secondary role to light intensity in promoting photosynthesis. However, it is possible that spectral quality played a role in the photoacclimation (photoadaptation) of the symbiotic zooxanthellae.
Discussion
The PAM fluorometer is a relatively easy instrument to use. Its microprocessor does all the work by command from keystrokes. However, explaining why the zooxanthellae reacted as they did to the different light spectra requires some detective work.
Photoadaptative mechanisms of Fungia species and their zooxanthellae have been studied and were reported by Masuda et al (1992). These scientists found chlorophyll a content of zooxanthellae within Fungia repanda and F. echinata increased with depth (though the chlorophyll a content per surface area of the coral did not change). A decrease in coral host tissue mass was also noted, and the compensation point (the point at which the oxygen demand of the coral host and symbiotic zooxanthellae is satisfied by oxygen produced by photosynthesis) also dropped. It is believed that the reduced amount of coral host tissue explains the lower compensation point, that is, less tissue means less oxygen required for life support. Masuda et al. also reported zooxanthellae of these Fungia adapted to low light intensity by altering the size of the Photosynthetic Unit (or PSU, defined as a single Photosystem I and a single Photosystem II, plus all associated light harvesting compounds working together – Kirk, 1994). Hence, these corals adapted to light intensity through alteration of host tissue mass and PSU size. According to this adaptation strategy, maximum rate of photosynthesis would decrease with depth, and respiration rates will drop accordingly. The report by Masuda et al. does demonstrate that at least some Fungiids have a remarkable ability to adapt to light fields at different depths, and it is possible that many, if not all, Fungiids use these photoadaptive mechanisms.
Evidence exists that other corals use different adaptation mechanisms. Mate (1993) reported that chlorophyll concentration per surface area and per zooxanthellae increased with depth in Montastraea cavernosa, Siderastrea sp., and Stephanocoenia sp. It should be noted there was no significant increase in number of zooxanthellae per surface area. Kirk (1994) references information about Stylophora pistillata, whose zooxanthellae increased chlorophyll a content and also increased the size of the PSU in shaded conditions. However, this explains how corals’ zooxanthellae could adapt to changing light quality and/or intensity – not why.
So, what might explain the similarities in ETR between the photoadapted corals? Fortunately, journal literature exists about coral photoadaptation, and even more importantly, chromatic adaptation by zooxanthellae ( Symbiodinum spp.). Early research papers reported results of coral transplantation experiments, where corals were moved to deeper, or shallower, depths and survival rates were observed (Dustan, 1982). Dustan’s experiments demonstrated that coral colonies transplanted from 30 to 15 m suffered from reduced growth, algal bleaching and high mortality rates. Although spectral quality was considered in Dustan’s paper, no opinions were made about chromatic adaptation, and a conclusion states: “…deep algae are damaged by the high light intensities that occur in the shallows.” (Dustan does show data that demonstrates a general decrease of blue and red wavelengths with increasing depth. And, as a point of reference, Dustan observed high survival rates of corals transplanted from 10 to 30 m.) Two years after the publication of Dustan’s paper, Kinzie et al. (1984) reported results of zooxanthellae chromatic adaptation experiments. Replicate experiments by Kinzie and Hunter (1987) corrected some errors advanced in the earlier work of 1984. Interestingly, the 1987 paper advanced a theory that the amount of incident red light is the parameter that regulates zooxanthellae cell density and, hence, pigment content. This hypothesis (confirmed by results of their experiments) states that corals subjected to increasing amounts of red light will reduce the number of zooxanthellae cells until totally bleached. These scientists used natural sunlight and filters to transmit a broad red bandwidth (~600 to 800 nm). (Note: Coral bleaching has also been seen at a narrower red bandwidth produced by an artificial light source – a light-emitting diode (LED) – Riddle, in press). Why might red light induce a reduction of zooxanthellae and/or photopigments? Why does red light seem to be the controlling bandwidth for the content of zooxanthellae photopigments? An answer lies in the remarkable process we know as photosynthesis.
Photosynthesis, as we know, is the link between the inorganic and organic worlds. Light energy absorbed by photopigments leads to production of oxygen, simple sugars, amino acids and fatty acids. These photochemical processes are dependent upon two distinct photosystems acting in unison, and are called, appropriately enough, Photosystem II (PS II) and Photosystem I (PS I). Very simply, these photosystems are dependent upon each other. There must be a balanced energy distribution between PS II and PS I (since Photosystem II produces an oxidant and PS I a reductant, and makes possible the electron donor/acceptor shuttle between the systems). In photosynthetic corals, this balance is probably achieved through control and/or alteration of photosynthetic pigment content of zooxanthellae (Kinzie et al., 1984). For instance, the accessory pigment peridinin transfers harvested light energy to chlorophyll a (with efficiencies ranging from 88% to >95%, see Damjanovic et al., 2000) and hence to PS II’s reaction centers containing Pigment 680. On the other hand, the carotenoid beta-carotene transfers its harvested energy to chlorophyll a and PS I (which contains Pigment 700). Increased absorption of light energy above 680 nm by coral zooxanthellae is associated with aggregated forms of chlorophyll a and PS I’s Pigment 700 (Titlyanov et al., 1980). Corals adapted to a certain light field will, when subjected to different light quality, adjust to the new light environment, or, under extreme circumstances, will bleach. It is possible that an imbalance of energy distribution could destroy PS II within a zooxanthella, thereby shutting down photosynthesis. Iglesias-Prieto (1997) discusses the destructive effects of accumulated electrons within PS II. The inability of PS II to transfer electrons effectively increases excitation pressure on the reaction center, and can occur at any light intensity. Brown (1997) discusses depressed rates of electron transfer between PS II and PS I before reduction in zooxanthellae density (number) is noted. Photosynthesis is effectively stopped when PS II fails to function. As a footnote, energy absorbed by PS II pigments can transfer to PS I to correct an electron imbalance in an effect known as ‘spillover.’ Spillover is known to occur in dinoflagellates (Schofield et al., 1996). In other words, a mechanism exists to maintain rates of photosynthesis if a coral is subjected to a suddenly altered spectrum. However, spillover seems only to occur when it is PS I that is under-stimulated: Spillover from PS I to PS II does not occur. Since PS I and PS II accessory pigments absorb essentially the same light wavelengths except for ~680 nm and above, spillover might occur when light is deficient in far-red wavelengths. This is likely not the case with many lamps used as light sources for aquaria. This is an important point – The red bandwidth and intensity of artificial light sources could over-stimulate PS I, thereby preventing any energy absorption – spillover – from PS II. Photosystem II must then ‘dump’ energy away from its reaction centers to avoid destruction by oxygen radicals overwhelming an enzymatic defense. Fluorescence or non-radiant heat transfer can dissipate energy harvested by photopigments, or certain pigments can cause dynamic photoinhibition, where light energy is diverted from PS II altogether. If none of these protective measures are effective, PS II is damaged and photosynthesis slows, or in extreme cases, stops completely.
If we examine the action spectrum for zooxanthellae, we see that blue and red wavelengths are preferentially absorbed by photopigments. Indeed, we need only to look at absorption of zooxanthellae photopigments. Chlorophyll a absorbs energy most strongly at 420 nm and 660 nm, chlorophyll c2 at 449 nm and 625 nm and beta-carotene at 425 nm, 450 nm and ~480 nm. Specialized forms of chlorophyll located in the reactions centers absorb light at 680 nm, and 700 nm. Peridinin (a carotenoid) absorbs mostly blue light (most strongly at about 450 nm and 490 nm), but extends its absorption range into the green portion of the spectrum. The xanthophyll diatoxanthin also absorbs blue light energy. This is an important consideration, since diatoxanthin is involved in dynamic photoinhibition through its light-activated (and reversible) conversion to diadinoxanthin. As such, zooxanthellae are protected against super-saturating light intensity by the absorption of blue light – if blue light energy is shunted away from photosystems, photosynthesis is regulated to ‘safe’ levels.
It is important to note that photosynthesis-quenching pigments (such as diatoxanthin) do not absorb red wavelengths – dynamic photoinhibition involves only absorption and dissipation of short-wave radiation such as violet and blue bandwidths. A water column rapidly attenuates red wavelengths (something on the order of 40% in the first meter of the most optically transparent seawater, Jerlov Oceanic Type 1 – 1976) so many corals would not need a specialized pigment to divert red light energy away from photosystem reaction centers. If we disregard the possibility of red-reflective or red-absorbing (fluorescent) pigments acting as photoprotectants, then zooxanthellae/corals do not possess a pigment to protect them from photo-reactive red light energy. It seems the mechanism they possess is a reduction of zooxanthellae and/or photopigment content, possibly leading to total bleaching. Results from recent experiments suggest that degrees of pigment reduction/bleaching are related to red light intensity of artificial light (
Riddle – in press).
Evidence does not discredit the notion that red light – at lower intensity – is a factor regulating zooxanthellae pigment content, that is, zooxanthellae might adjust pigment content until equilibrium between PS II and PS I is obtained. If the red light intensity is great enough, photoacclimation may not be possible, and bleaching is a last ditch effort for survival.
Red light content of each of the lamps used in this experiment was remarkably similar. Figures 5 and 6 illustrate the spectral content of Lamp 10K and Lamp 50K; Table 1 lists the content in tabular form.
Color | Sunlight | Lamp 10K | Lamp 50K |
---|---|---|---|
Violet (400 – 430 nm) | 4 | 12 | 8 |
Blue (431 – 480 nm) | 13 | 16 | 38 |
Green-Blue (481 – 490 nm) | 4 | 1 | 3 |
Blue-Green (491 – 510 nm) | 10 | 3 | 9 |
Green (511 – 530 nm) | 10 | 3 | 15 |
Yellow-Green (531 – 570 nm) | 19 | 29 | 15 |
Yellow (571 – 580 nm) | 5 | 16 | 3 |
Orange (581 – 600 nm) | 8 | 7 | 3 |
Red (601 – 700 nm) | 27 | 12 | 11 |
PPFD was measured periodically during the 30-day period with a Li-Cor Model 189 quantum meter with 2-pi submersible sensor. The meter was calibrated for ‘water’ measurements. Crude estimates of lamp spectral qualities were made during the 30-day photoadaptation process using the Li-Cor quantum meter and red, green, and blue glass band pass filters. Spectral stability is not a trait of some metal halide lamps (especially those of higher Kelvin ratings), however the red portion of the output of both lamps was remarkably similar for Lamp 10K and Lamp 50K, averaging 19.1 and 21.1 micromol per square meter per second, respectively. PPFD increased from Lamp 50K during the course of the experiment (an anomaly – an exact explanation is beyond the scope of this article) and the amount of photosynthetically active radiation generated by this lamp (as measured at the coral’s surface) averaged 65.5 micromol per square meter per second. PPFD generated by Lamp 10K averaged 57.9 micromol per square meter per second. See Figures 7 and 8.
It is possible that a similar amount of red radiation generated by the two lamps was the prevailing factor in ultimately determining the photopigment content of zooxanthellae during the 30-day photoadaptation period. We did not examine pigment/zooxanthellae content but instead relied upon what ultimately matters most – quantum yields of photosystems – to estimate rates of photosynthesis.
Light intensities used in these experiments were likely sub-saturating (based on compensation and saturation points of Fungia specimens reported by Masuda et al., 1992, and combined with my information gathered from WHEA’s coral tanks). Since photosynthesis/irradiance curves flatten when light intensity is at or above the light saturation point (given that no photoinhibition is occurring), measuring the ETR under this condition (saturating intensity) would not generate the sort of information sought in this experiment. However, one should view these ETRs as relative only to the particular spot of the coral. Base line ETRs were collected for both corals when illuminated by each of the two distinctly different light sources. This information (not shown) was ultimately of little use for comparative purposes.
It is apparent that rates of photosynthesis can vary greatly across the surface of a coral, even a relatively flat specimen such as Fungia (this should not be surprising, as photosynthetic rates are known to vary across the surfaces of leaves; this phenomena has also been previously reported to occur in corals – see Warner et al., 1999). However, the intent of this experiment was not to demonstrate variations in photosynthesis across surface area, but to report rates of photosynthesis in a given spot when illuminated by a light source to which the coral has adapted, and by one to which it has not adapted.
It was quite accidental that the lamps chosen for this experiment contained similar amounts of red light energy. Future experiments should utilize lamps that generate dissimilar amounts of red light energy. Experiments are underway to determine the effects of bundled LEDs producing essentially red, and blue, monochromatic light. For now, the results of this experiment are inconclusive in answering the question of intensity vs. spectral quality in promotion of photosynthesis within photoacclimated coral zooxanthellae. However, evidence does not discredit the theory that red light is a factor in determining ultimate rates of zooxanthellae photosynthesis.
Part II: Effects of Water Motion on Zooxanthellae Photosynthesis
Water motion has long been recognized as an important factor in the successful maintenance of reef aquaria. However, the importance of water movement is often overlooked in favor of lighting requirements. The result of a simple experiment suggests that low water motion can limit photosynthesis in zooxanthellae of captive corals. A theory is advanced in the Discussion section.
Procedure
A small Lobe Coral ( Porites lobata ) colony was placed in a 3-gallon aquarium that was aerated with a small air stone, which provided water circulation within the aquarium. A specially built jig held the fiber optic sensor of a Walz Pulsed Amplitude Modulation (PAM) fluorometer at 60-degree angle close to the coral colony. The fluorometer was programmed to assess the quantum yield of photosynthesis at various light intensities (using the built-in actinic LED light source). One set of readings were taken, and the air pump was turned off, and the coral was allowed to again ‘dark adapt’ for 20 minutes before another set of quantum yield measurements were made (this time ‘without’ water motion). The air pump was turned on, the coral allowed to ‘dark adapt’ and a set of yield measurements were made ‘with’ water movement. These procedures were repeated several times.
The quantum yield measurements were downloaded from the fluorometer into an Excel worksheet. Estimates of actinic lamp intensity provided by Walz for use with the fiber optic cable were used to calculate relative electron transport rates. These light intensities ranged from zero to 813 μmol/m2/sec.
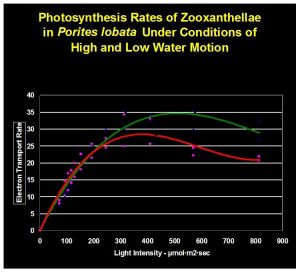
Figure 9. The green line indicate rate of photosynthesis ‘with’ water motion. The red line is the photosynthetic rate ‘without’ water movement.
Results
The results are shown in Figure 9. They suggest that low water motion can limit photosynthesis even as light intensity increases.
Discussion
It is apparent that lack of water motion impacted photosynthesis in a negative manner. Though determination of the exact cause of photosynthesis inhibition was beyond the scope of this experiment, we can speculate that stagnant water of a thickened boundary layer limited diffusion of an element required for photosynthesis. Divergence of trend lines is apparent at about 200 μmol/m2/sec, suggesting that a critical requirement of photosynthesis is not being met at light intensity exceeding this amount when water motion is practically non-existent.
The practical implications for hobbyists are clear. While much has been written on the benefits of proper water motion in the arenas of food delivery, waste removal, element diffusion, etc., very little has been discussed on the effects of insufficient water movement within artificial environments. The results of this simple experiment suggest several things. Perhaps most importantly is the realization that light intensity alone is not enough to promote maximum rates of photosynthesis. As the demands of photosynthesis increase (an unknown from the results of this experiment), delivery of these elements might become limited by insufficient water motion. By simply adding an additional powerhead (or other device), the full benefits of a lighting system might be realized. Conversely, additional water movement could potentially make a marginal lighting system adequate.
Photoinhibition is apparent in both trend lines. The light intensity is very high (800 μmol/m2/sec) at which photoinhibition is most pronounced. One should bear in mind that this experiment was conducted with the PAM fluorometer’s internal actinic light set at minimum duration. Photoinhibition will likely occur at lower light intensities when photosynthesis is allowed to ‘develop a full head of steam.’
Future use of PAM fluorometry will generate much information, and will likely answer many existing questions. Quantification of required water motion for ‘difficult’ adult coral colonies (such as Pocillopora meandrina ) is now within our reach. Effects of ultraviolet radiation can be effectively demonstrated. Interestingly, qualification of food sources for non- photosynthetic corals is also possible with this instrument. In short, answers to many of the debating points among hobbyists will soon have an answer.
I will begin work on November 3, 2003 on a project designed to unlock the mysteries of coral coloration. This ambitious project is partially funded by a research grant from the National Oceanic and Atmospheric Administration (NOAA). Work is projected to take 13 months to complete; however, most of the results will be available for my presentation at Boston’s MACNA in 2004. I hope to see you there.
Acknowledgements
My thanks go to Brian Uyeda of HelloLights (http://www.hellolights.com) for supplying the metal halide lamps used in experiments of Part I. I would also like to thank Bill Woerner of West Hawaii Explorations Academy for allowing me use of the facility (and for paying the electric bill for the 30-day acclimation period!). Special thanks go to Sara Peck of University of Hawaii Sea Grant (West Hawaii) for her invaluable assistance.
References
- Brown, B., 1997. Coral bleaching: Causes and consequences. Proc. 8th Int. Coral Reef Symp., Panama. 1:65-74.
- Damjanovic, A., T. Ritz and K. Schulten, 2000. Excitation transfer in the peridinin-chlorophyll-protein of Amphidinium carterae. Biophysical Journal, 79: 1695-1705.
- Dustan, P., 1982. Depth-dependent photoadaptation by zooxanthellae of the reef coral Montastraea annularis. Mar. Biol., 68:253-264.
- Iglesias-Prieto, R., 1997. Temperature-dependent inactivation of Photosystem II in symbiotic dinoflagellates. Proc. 8th Int. Coral Reef Symp., Panama. 2: 1313-1318.
- Jerlov, N., 1976. Marine Optics. Elsevier Oceanography Series, Elsevier Sci. Publ. Co., New York. 231 pp.
- Kinzie, R.A., 1993. Effects of ambient levels of solar ultraviolet radiation on zooxanthellae and photosynthesis of the reef coral Montipora verrucosa. Mar. Biol., 116:319-327.
- Kinzie, R.A., P.L. Jokiel and R. York, 1984. Effects of light of altered spectral composition on coral zooxanthellae associations and on zooxanthellae in vitro. Mar. Biol., 78:239-248.
- Kinzie, R.A. and T. Hunter, 1987. Effect of light quality on photosynthesis of the reef coral Montipora verrucosa. Mar. Biol., 94: 95-109.
- Kirk, J.T.O., 1994. Light and Photosynthesis in Aquatic Ecosystems. Cambridge University Press, Cambridge. 509 pp.
- Masuda, I., M. Goto, T. Maruyama, and S. Miyachi, 1993. Photoadaptation of solitary corals, Fungia repanda, F. echinata, and their zooxanthellae. Proc. 7th Int. Coral Reef Symp., Guam. 1: 373-378.
- Mate, J.L., 1993. Variations in chlorophyll concentration and zooxanthellae density with depth in Caribbean reef corals of Panama. Proc. 7th Int. Coral Reef Symp., Guam. I: 382 (abstract).
- Saxby, T., W. Dennison and O. Hoegh-Guldberg, 2003. Photosynthetic responses of the coral Montipora digitata to cold temperature stress. Mar. Ecol. Prog. Ser., 248:85-97.
- Schofield, O., B. Prezelin and G. Johnsen, 1996. Wavelength dependency of the maximum quantum yield of carbon fixation for two red tide dinoflagellates, Heterocapsa pygmaea and Prorocentrum minimum (Pyrrophyta): Implications for measuring photosynthetic rates. J. Phycol., 32, 574-583.
- Schreiber, U., 1997. Chlorophyll Fluorescence and Photosynthetic Energy Conversion. Heinz Walz GmbH, Effleltrich. 73 pp.
- Titlyanov, E.A., M.G. Shaposhnikova and V.I. Zvalinskii, 1980. Photosynthesis and adaptation of corals to irradiance. I. Contents and native state of photosynthetic pigments in symbiotic microalga. Photosynthetica 14 (3): 413-421.
- Warner, M., W. Fitt, and G. Schmidt, 1999. Damage to Photosystem II in symbiotic dinoflagellates: A determinate of coral bleaching. Proc. Natl. Acad. Sci. USA. 96:8007-8012.
0 Comments